Plasmonic and 2-D materials for sunlight-to-chemical energy conversion
Plasmonic and 2-D materials are revolutionizing the quest for sustainable energy, merging cutting-edge science with environmental impact. Plasmonic materials amplify energy transfer using localized surface plasmon resonances, while 2-D materials offer unmatched surface area and exceptional electronic properties. By joining forces, these innovative materials unlock new possibilities for solar-driven chemical transformations, paving the way toagreener future by reducing dependence on fossil fuels and powering next-generation green energy solutions.
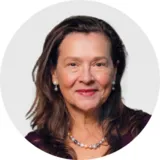
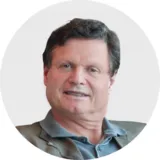
Focus Group: Sustainable Photocatalysis Using
Plasmons and 2-D Materials
Prof. Naomi J. Halas (Rice University), Alumna Hans Fischer Senior Fellow (funded by Cluster of Excellence e-conversion) | Prof. Peter Nordlander (Rice University), Alumnus Hans Fischer Senior Fellow (funded by Cluster of Excellence e-conversion) | Dr. Olivier Henrotte (LMU), Postdoctoral Researcher | Jan Schabesberger (TUM), Doctoral Candidate | Hosts: Prof. Jonathan Finley, Prof. Ian Sharp (TUM), Prof. Emiliano Cortés (LMU)
Overall scientific concepts and goals
The urgent need for sustainable energy solutions has driven significant interest in photocatalysis, a process that utilizes light to drive chemical reactions, offering a pathway to clean energy production and environmental remediation. Conventional photocatalysts often suffer from low efficiency due to limited light absorption and charge recombination. To address these challenges, this project focuses on integrating plasmonic and two-dimensional (2-D) materials to advance photocatalytic systems.
Plasmonic materials, such as noble metal nanoparticles, exhibit localized surface plasmon resonances (LSPRs) that enable efficient light harvesting and energy transfer. When coupled with 2-D materials such as graphene, transition metal dichalcogenides (TMDs), or hexagonal boron nitride, the combined system benefits from enhanced charge separation, large surface areas, and tunable electronic properties. Together, these materials hold the potential to significantly boost the efficiency and sustainability of photocatalytic reactions.
The primary goal of this project is to understand the fundamental mechanisms of photocatalysis and light-to-chemical energy conversion on plasmonic materials and 2-D materials. By advancing the fundamental understanding of light-matter interactions and charge dynamics in these systems, this research aims to bridge critical gaps in the field and provide scalable solutions for global energy and environmental challenges.
This work is situated at the intersection of materials science, nanotechnology, and energy research, addressing pressing issues of energy security and environmental sustainability. The outcomes will not only advance photocatalytic technologies but also contribute to broader efforts in sustainable energy and green chemistry.
Figure 1
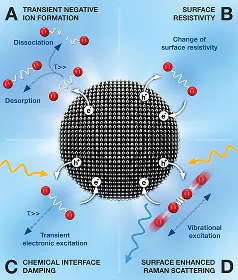
Summary of research work carried out and outcomes
The Focus Group Sustainable Photocatalysis Using Plasmons and 2-D Materials focused on two fundamental areas for sustainable photocatalysis: plasmon-driven energy transfer and 2-D materials for light-to-chemical energy conversion.
Plasmon-driven energy transfer and operando spectroscopy
Understanding the mechanisms of metal-molecule energy transfer in plasmonic materials is critical for advancing sustainable photocatalysis. Plasmonic materials, such as gold and silver nanoparticles, exhibit unique optical properties that allow them to harvest light efficiently through localized surface plasmon resonances (LSPRs). When light interacts with these materials, it excites electrons, creating high-energy carriers that can transfer energy to nearby molecules. This energy However, the efficiency of these processes depends on precise control of energy transfer pathways, including hot electron injection, Förster resonance energy transfer, and thermal effects. Therefore, during the Fellowship we published a review focused on the mechanisms of plasmon-driven chemical reactions (see Fig. 1). We have shown that several mechanisms that involve energy transfer or exchange between an electron, a nanoparticle, and an adsorbed molecule can be rationalized under the same fundamental framework of resonance and non-resonance electron transfer. Thus this work bridges the field of plasmon driven photocatalysis with the more classical field of surface science, enabling the design of more efficient plasmonic catalysts.
This work led us to the idea that there is an underlying connection between the effect of adsorbed molecules on the electrical and optical properties of metallic nanostructures, a work which is in preparation for publication. The basic idea is that adsorbed molecules on metal surfaces disrupt the flow of electrons, increasing the electrical resistance of the metal. This effect is physically similar to the case when metal electrons are excited through light and can transfer energy to
adsorbed molecules. Therefore, our work advanced the understanding of the interaction between plasmonic materials and adsorbed molecules, with the end goal of using plasmonic materials for industrially relevant processes such as clean hydrogen production.
A big challenge in developing more efficient plasmonic photocatalysts is monitoring their activity during the photocatalytic process, i.e., operando. A very powerful tool for tracking plasmon-driven chemical reactions in operando conditions is surface-enhanced Raman spectroscopy (SERS). This method uses light to investigate the chemical composition of molecules and can therefore be used to track every step of a photocatalytic reaction. During our research work, we reviewed the use of SERS in all areas of catalysis and energy conversion, from photocatalysis to battery development (see Fig. 2). Therefore, this work provides an exhaustive view of operando monitoring of catalytic materials and chemical processes and highlights future areas of interest in energy conversion, such as using artificial intelligence (AI) to accelerate material discovery.
Figure 2
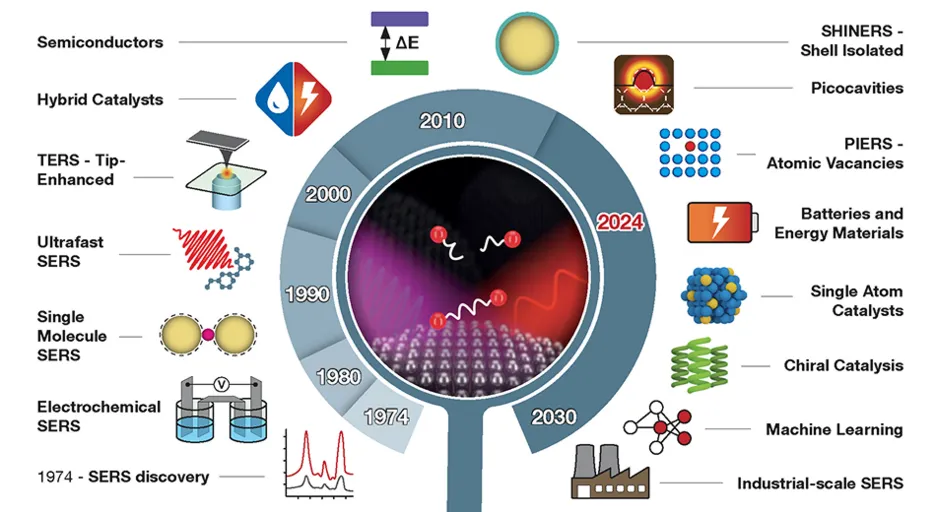
real -time monitoring of energy conversion processes. In this figure, we summarize a selection of the most important milestones and developments of SERS in the field of catalysis over the last 50 years. © Stefancu, A. et al. Impact of surface enhanced raman spectroscopy in catalysis. ACS Nano 18, 43, 29337–29379 (2024).
Monitoring the photochemical reactivity of 2-D materials
Two-dimensional transition metal dichalcogenides (2-D TMDs) are promising material platforms for optoelectronics, sensing, and quantum information technologies, showing significant potential for groundbreaking applications. They also hold immense potential for photocatalysis, due to their earth-abundance, strong light-matter interaction, and high surface-to-volume ratio, which means they can host many active sites for chemical reactions. However, progress has been limited due to an incomplete understanding of their photocatalytic reactive sites and their reactivity.
Therefore, it is essential to identify active chemical sites and monitor their reactivity under appropriate operando conditions, using advanced approaches that can probe these aspects. Employing this unique, performance-oriented feedback, the material synthesis can be optimized regarding morphology, dopants, and other defects. This will provide a deeper understanding that will lead to novel photocatalyst design, fully leveraging the tunability of 2-D TMDs to develop next-generation materials and set new benchmarks in efficiency and sustainability.
To accomplish this goal, we developed a novel operando imaging method capable of identifying the spatial distribution of reactive sites for oxidation and reduction on a 2-D TMD: an MoS2 monolayer (ML), using light as the sole external driving force (see Fig. 3). We observed the effects of the electronic properties of the material, electron/hole transport, and lateral confinement of photocarriers on chemical reactivity. Unlike other imaging techniques that have been recently utilized to study photocatalytic systems, this new imaging method enables the direct mapping of efficiency with high spatial resolution (~200 nm) and allows the local measurements of hydrogen generation under light excitation for the first time.
The separation and transport of charge carriers during photocatalysis are also critical factors in solar-driven devices. Building on insights from our study, we explored strategies to enhance charge carrier mobility on 2-D platforms. We observed a significant improvement in the efficiency of MoS₂ under visible light, with charge extraction increasing by 5 to 30 when connected to a conductive material. Notably, this enhanced performance extended over distances of several hundred micrometers from the connection point.
These findings offer a clear path toward designing highly efficient sunlight-powered devices by improving charge transport over long distances and optimizing photocatalytic performance. Such advancements could significantly impact solar energy conversion technologies, hydrogen production, and sustainable energy systems.
Figure 3
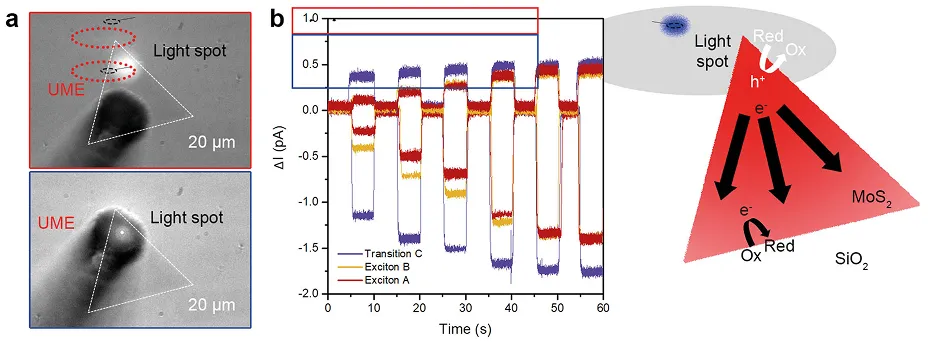
photogenerated charge carriers (oxidation: holes, blue region; reduction: electrons, red region). © Henrotte, O. et al. Spatially resolved photocatalytic reactive sites and quantum efficiency in a 2D Semiconductor. Submitted, 2025.
Synthesis of 2-D materials for photocatalysis
Using this insight into the photocatalytic activity at the microscopic level, the epitaxial growth of the 2-D material can be tuned for increased catalytic performance. As line or point defects can trap photoexcited charge carriers and act as reactive sites, material parameters such as morphology, layer number, or dopants will greatly influence the overall performance. We worked on understanding the epitaxial growth of different 2-D materials using chemical vapor deposition (MoS₂) and molecular beam epitaxy (InSe, GaSe) as better alternatives to the dry stamping transfer technique, aiming at crystals of more reproducible interface quality due to the controlled deposition process. Connected to the MBE system, we developed an all-in ultrahigh-vacuum optical (Raman, photoluminescence, differential reflectance) analytic setup to characterize 2-D material properties in pristine conditions. As a next step, we will use this capability to analyze MBE-grown layers on top of plasmonically active structures and test the electronic properties of the heterostructures, all crucial for photocatalytic applications. A challenge in this respect is optimizing growth recipes for substrates that can yield high-quality crystals but are also suitable for plasmonic applications.
Future related research
In summary, our research project achieved its initial goal of understanding better light-to-chemical energy conversion in plasmonic systems and in 2-D materials, specifically MoS2. These advances will allow us to translate plasmonic and 2-D materials into practical applications for clean hydrogen generation or CO2 reduction. One promising approach based on our results is to combine plasmonic and 2-D materials, forming hybrid reactors that would combine the ability of plasmonic nanoparticles to absorb light and the reactive sites of the 2-D material.
In close collaboration with Dr. Andrei Stefancu (LMU).
Selected publications
- Stefancu, A., Halas, J.N., Nordlander, P. & Cortes, E. Electronic excitations at the plasmon–molecule interface. Nat. Physics 20, 1065–1077 (2024).
- Stefancu, A. et al. Impact of surface enhanced raman spectroscopy in catalysis. ACS Nano 18, 43, 29337–29379 (2024).
- Henrotte, O. et al. Spatially resolved photocatalytic reactive sites and quantum efficiency in a 2D Semiconductor. Submitted, 2025.
- Stefancu, A. et al. Optical and electrical probing of plasmonic metal-molecule interactions. To be submitted, 2025
- Henrotte, O. et al. Optimizing charge transport in a 2D Semiconductor for enhanced photocatalysis. In preparation, 2025.
Full list of publications:
Prof. Naomi J. Halas
Prof. Peter Nordlander