In Focus:
Probing Perovskites
Researchers from Munich and Oxford are exploring novel materials that could boost solar cell efficiency. Laura Herz, David Egger, and Thomas Bein form a dynamic “research triangle” combining expertise in condensed matter physics, theoretical physics, and physical chemistry to understand and optimize energy conversion.
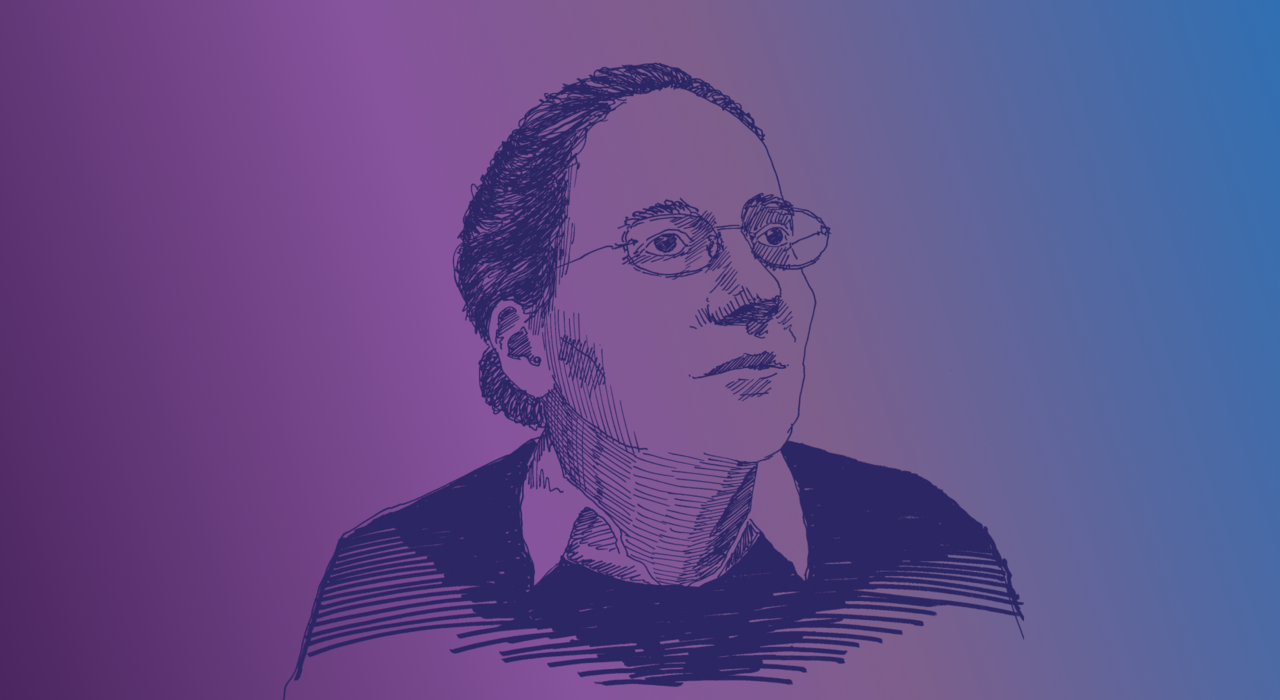
Can global, long-term environmental, economic, and security challenges be addressed, or at least mitigated, by probing artificial materials on a femtosecond time scale? The answer might be yes if this research contributes to a more rapid switch to energy production from renewable sources such as sunlight. Slowing global warming and adapting to its impacts will depend on drastically cutting greenhouse gas emissions while reducing dependence on fossil fuels. These same steps could make economies and nations less vulnerable to any disruption of gas and oil supplies.
The TUM Institute for Advanced Study Focus Group on Optoelectronic Properties of Perovskite Semiconductors is exploring the physics and chemistry of novel materials that have the potential to boost the efficiency of solar cells. The group is led by Hans Fischer Senior Fellow Laura Herz, a professor of physics at the University of Oxford, where she directs the Semiconductors Group at the Clarendon Laboratory and serves as associate head of Oxford’s Mathematical, Physical, and Life Sciences Division. Her Munich collaborators are TUM-IAS Rudolf Mößbauer Tenure Track Assistant Professor David Egger and Professor Thomas Bein of LMU, both of whom are principal investigators in the DFG-funded Cluster of Excellence “e-conversion.”
For an inside view of fundamental studies that could open the way for the development of more efficient solar cells – enabling further expansion of photovoltaics within the global energy mix – science journalist Patrick Regan spoke with Laura Herz via videoconference in February 2023. Their conversation has been edited for length and clarity.
Q: Photovoltaics technology, in real-world products, has come a long way in terms of cost, performance, reliability, and market acceptance, especially during these past two decades. That’s roughly the same time in which researchers discovered and began exploring the potential of so-called perovskite materials for solar cells. Would development and commercialization of perovskite solar cells represent incremental progress or something more like a breakthrough?
People are now seeing solar energy in reality. They’re installing solar panels everywhere. You can see how much electricity even Germany or the UK can generate from solar on a good day. Twenty or thirty years ago we were told this could not happen because the weather was awful, and it was too expensive. Now we’re installing it and reaching a terawatt worldwide – and why is that? Partly because the cost of silicon solar panels has fallen massively over the past decades and keeps falling. A lot of that is caused by mass production. Manufacturing has moved to China, where they have ruthlessly cut costs and have been supplying us with ever cheaper panels.
So why perovskites at this stage? Because the cost of the actual silicon solar cell has fallen so much, what now matters most is the on-cost, everything that’s needed to actually plug that cell into the grid. In a domestic installation that would mean putting it on your roof, reconstructing that roof. You also have to add electrical components, such as an inverter, because ultimately your solar cell gives you a DC low voltage, and you have to plug it into the grid, which is 240-volt AC. All of that now forms most of the cost of the installation. This means that if you want to further reduce the cost of solar energy generated, it’s not really about reducing the cost of the cell, but about increasing its efficiency. The way you need to look at cost is how much “watt peak” – a measure of peak power – you return on that whole investment. So if you’re improving efficiency, you’re returning something more across the whole installation. If you just lower the cost of the cell, you’re lowering the cost of an element that’s already not contributing much to that overall cost. And that’s why solar cell research has now become all focused on efficiency.
And silicon has more or less peaked in terms of efficiencies and can’t be improved much further. Silicon is a great material. It’s made essentially from silicon dioxide, sand, so it’s very abundant. But it’s got what’s called a fixed bandgap: you can’t tune it. If you think of a semiconductor, you essentially have a material that absorbs light, photons that have a certain energy, and all the energy above that bandgap will be absorbed. The bandgap of silicon is just over one electron volt, and so every photon that has an energy above that will be absorbed, and anything below that will pass through.
Say there’s a photon coming along, and it’s got three electron-volt energy. And the bandgap of the light-absorber material is one electron volt. These two electron volts extra, that extra bit of energy, will be lost in the form of heat. So that’s a waste, a huge waste.
To avoid such heat loss we move beyond solar cells with single absorber layers, by making multi-junction cells: We put one semiconductor absorber layer on top of another, with different bandgaps. The absorber layer facing the sun grabs the highest-energy photons first. Anything below that passes through, hits the next layer, which grabs the next lot, and the remainder passes through and is incident on the bottom layer. So if you go for a triple-junction stack, you can roughly double solar-cell efficiency with respect to silicon. That’s because you’re able to harvest energy now that otherwise would be lost as heat.
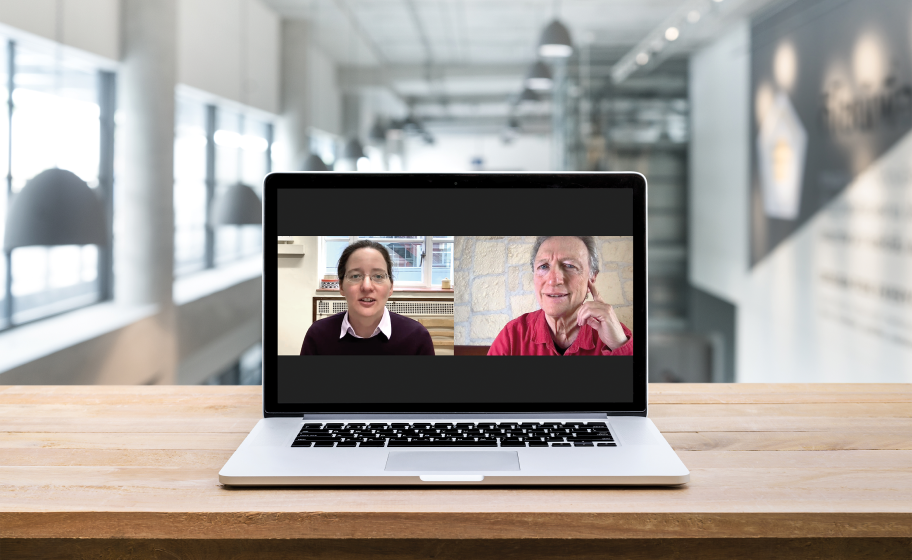
Q: And stacking cells with different bandgaps depends on creating new materials and understanding their behavior. Does this bring us closer to your research focus?
We look at the fundamental processes that underpin how these materials work. If you think about it, we start with sunlight, and turn that into electricity. My research is asking what’s happening in between and how we can optimize that process. Light is not charged – it’s made of these little particles called photons, and they’re not charged – so how do you end up with moving charges, electricity? At first, the absorber material, the semiconductor, has to capture that photon efficiently. Then the initial excitation the photon has created has somehow to be turned from a neutral entity into a pair of positive and negative charges. That’s the process. How does that happen?
If you excite this semiconductor, and you ultimately create something that’s an electron and a hole – a hole is like a missing electron – those two attract each other. So how come they move apart to make that current? Are there any obstacles they encounter in their path out of the absorber layer? Are these obstacles fundamental in nature, for example, because these charges “bump” into the ions that hold the material together? How can we describe and understand these processes to then inspire the creation of even better absorber materials? These are the questions that we are trying to figure out.
Q: How do you probe such fundamental phenomena?
We have to simulate the whole process. We use ultrafast laser pulses to trigger absorption of photons, and then we ask: Is any neutral particle forming? Does it turn itself into charges? Are they moving freely? How fast are they moving? Do these charges maybe localize somewhere? Are they being trapped by some material defects? Do these charges make it out to collection contacts? And what fundamentally is underpinning each of these steps? Incrementally we can probe all of these processes and disentangle them. Ultimately, we can understand what makes a material work well in a solar cell.
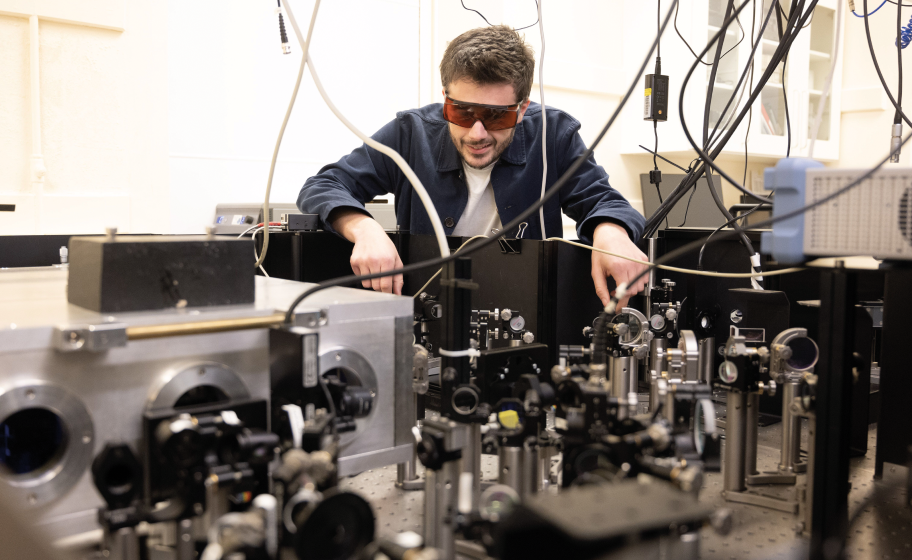
Q: So you’re probing these energy conversion phenomena from a lot of different angles, with some very sophisticated tools. Could you explain more about how you do it, and what kind of evidence comes out of these probes?
We do all of that work with advanced optical spectroscopy. It’s very important to us that we can probe what’s going on before people have even thought about optimizing their photovoltaic devices. My colleagues working on solar cell device fabrication work hard to try to figure out the best device architectures, the best extraction layers, and so on. If a new material just emerging is intrinsically incapable of producing a good solar cell, there’s no point in anyone optimizing a device structure. For these reasons it makes perfect sense for us to go in there with non-contact probes that are capable of just taking a thin-film layer and determining whether or not it’s a good candidate. And that means we’re exciting materials and probing properties with just a set of very short laser pulses that simulates the sunlight and then reading out properties a little while later.
Q: How short are the pulses?
Typically around 100 femtoseconds in duration. After that light has been absorbed, we can probe a number of different processes. For example, I talked earlier about how some of the light is lost in heat. So, for example, we can probe that heat loss by exciting with photons whose energy is much larger than the bandgap, and then watch the charges as they relax right to that band edge while losing energy in heat. And that heat is stuck in the system for a while, for example in the electron gas, or in the vibrations of the ionic crystal. In theory the heat stuck in the electron gas could still be extracted to generate electricity, if it remains long enough for the charges to be extracted. So we can probe how hot these charges remain and for how long. This could ultimately lead to so-called “hot-carrier solar cells” with very high efficiencies. That’s one of the measurements we can make at early times after photon absorption, for example, by measuring the energies of the photons that are emitted.
And then the next step might be to ask: How mobile are these charges? Can they move freely? The way we can probe this, again, is totally non-contact. We use another laser pulse to create what’s called a single-cycle terahertz pulse. That is a little bit like an AC electric field. You can think of it as being analogous to the electricity supply in your home at 50 hertz. Only it’s not 50 hertz, it’s a terahertz electromagnetic pulse, and that is shuffling these charge carriers around, moving them around, interacting with them and saying: Are you here, and is there anything that’s moving freely? Because that pulse is an electric field it interacts with charges, which modulates the terahertz pulse. So by reading out that terahertz pulse after it’s passed through we ask: Hey, have you encountered any free charge carriers in there? Have they been mobile? It’s a complex three-pulse experiment. We first have to generate that terahertz pulse with one short laser pulse.
Then we have to read out that terahertz pulse with another short laser pulse. And then we’re coming in with the simulated sunlight excitation pulse as well, in the visible part of the spectrum, and so we now have three laser pulses going.
“We look at the fundamental processes that underpin how these materials work. We start with sunlight, and turn that into electricity. My research is asking what’s happening in between and how we can optimize that process.”
Laura Herz
Q: In the same experiment?
In the same experiment. They’re all time-delayed in order for us to read all that out. It requires a very complex optical setup, home-built, home-thought-through. You can’t buy it off the shelf.
Q: Is your optical setup hidden in a deep, quiet basement somewhere outside the city of Oxford?
No, it’s right in the city, in an old heritage-listed building. One advantage is that the walls are one meter thick. Nothing moves, and we have very sophisticated vibration isolation mechanisms supporting the table, as well as an air conditioning unit that gives us precise control to keep the temperature stable.
Q: Let’s move on to the material in the spotlight, usually referred to simply as perovskite. What is it, and what advantages does it bring to this business of energy conversion?
It’s important to recognize that perovskite, in this context, is a material system rather than a single material. The name refers to a specific kind of crystal structure. The original perovskite is in fact one particular material (calcium titanate), but this label came to be used for all materials crystallizing in the same structure. When we talk about perovskite solar cells, we actually mean metal-halide perovskites. You can choose between different metals – it could be a bit of lead and a bit of tin – and between different halides, such as iodine or bromine. And you can mix them across these sites in the crystal structure, to have a random mixture of either iodine or bromine on a particular lattice point for example. This enables you to tune the bandgap of the semiconductor, which then allows you to tune the kind of photons that the material absorbs. For example, you could have a layer at the bottom of a multi-junction solar cell with a very narrow bandgap that has a mixture of tin and lead, and then you put another layer on top that’s got a mixture of iodine and bromine, and that way you have stacks of varying bandgaps to reduce the heat loss in the system, as I mentioned earlier.
In this perovskite structure you have a series of octahedra that are connected, which gives a certain flexibility. You can twist these octahedra with respect to each other. Depending on which atoms you place on the structure, the octahedra will tilt to accommodate. That is partly why you can tune these materials so easily: For example, you might add some bromine even though it’s smaller than iodine, and it will still fit in just as easily. The octahedra distort a bit differently, you get a different crystal structure overall, but the perovskite structure adapts. That allows bandgap tuning, and helps us access a wider, more flexible range of materials, which in turn allows the layering of materials with different bandgaps that makes it possible to reduce the heat losses in multi-junction solar cells.
You can’t do that with silicon. With silicon you are stuck where you are, with the one bandgap that it has. You can improve a little bit by making it a nice uniform block, and that will improve efficiencies, but then after that, it is what it is.
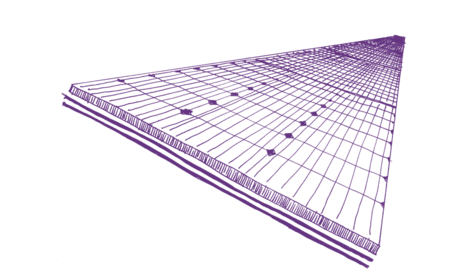
Q: Are metal-halide perovskites “nice uniform blocks” as well?
Actually, we published a paper in Science in 2020 reporting how we imaged the microstructure of these systems on an atomic scale for the first time. That hadn’t been done before because these materials tend to disintegrate under an electron beam. Our study showed that these materials can have beautifully periodic structures and that the interfaces are incredibly benign. We found really coherent grain boundaries, with atomic columns running towards each other in an almost perfect line right up to the interfaces. There’s hardly any amorphous material there. It’s beautifully formed.
This revealed one of the reasons why these materials work so well. The interfaces between individual crystal “bits,” or grains, are incredibly benign. And that’s perhaps relating back to the perovskite structure, because it is such a flexible structure that it alleviates strain. Normally you would see amorphous stuff forming, crystal lattice planes bending because of strain, dislocations and defects forming, but these grains and interfaces are beautiful. Another thing you often see in some materials is unwanted remnants of a precursor or formation of an oxide. One reason silicon is so successful is that silicon oxide forms such a benign interface with silicon. And in the Science paper we showed that metal-halide perovskite forms a fantastically benign interface with its precursor, lead iodide. No dislocations, just lattice planes merging straight into each other. That observation is probably a major reason why these materials work so well.
Q: That brings us to your TUM-IAS Focus Group, where your lab’s experimental prowess is coupled to cutting-edge research in theoretical physics and physical chemistry. Could you talk a bit about the synergies? Do you find, in practice, that the complementary approaches are mutually helpful?
It really is a very complementary assembly of expertise and exactly what you need to make progress quickly. On the one hand, Thomas Bein is synthesizing next-generation materials, some of which are perovskites, some perovskite-inspired. We’re always looking out for even better materials that might deliver higher stability, better efficiency, easier casting, and so forth. So they’re exploring a very wide space of materials with a very open mind. They are hard-core synthetic chemists who can make materials that perhaps no one else has made before.
On the other side, we have David Egger’s group, who are at the forefront of trying to develop the next generation of theoretical models that allow us to understand and predict how easily charges can move through the material. As I said before, charges have to be mobile, but they may be held back by interactions with the underlying lattice of ions that makes up the solid. It’s this ionic lattice that holds the crystal together, but of course the ions are charged, which will interfere with electrons and holes moving through. David has developed a new approach based on molecular dynamics simulations that explore such interactions, while being able to reflect properly this structural flexibility I talked about earlier, which really complicates matters in terms of making accurate predictions.
So my group is able to reveal experimentally the mobility of charges, and David Egger’s group can make accurate calculations, and Thomas Bein will provide novel semiconductors. By feeding that expertise and these processes back in an interdisciplinary triangle, we can both improve understanding and, in the long run, through iterations, improve and invent new materials themselves.
“By feeding that expertise and these processes back in an interdisciplinary triangle, we can both improve understanding and, in the long run, through iterations, improve and invent new materials themselves.”
Laura Herz
Q: Does David end up proposing experiments, or proposing things you might look for to test or confirm the theory?
Yes. We talk to each other frequently within this interdisciplinary triangle. He might say: I’ve got this theory, it predicts a particular trend, could you measure that? Or I might say: I’ve measured x, can your model explain that? And if that works, it’s fantastic. If it doesn’t work, then we’ll have to think again. Because it might just be that I can’t do those experiments, or that what I’m asking for would take so long to achieve computationally that we’ll be retired by the time we receive an answer. And similarly with synthesis, of course, I might say: Could you just put that particular atom on that site please? I don’t necessarily understand the intricacies of the synthesis, so they might have to tell me it’s sadly impossible. So again, we have to go back to the drawing board. These conversations are really important to have on a regular basis, and they give us many new ideas.
Q: Can you give an example of how this works?
At the moment we’re considering substitution on a specific site, for example a replacement of bismuth with antimony within a particular double perovskite. And so Thomas Bein’s group may synthesize a series of compounds gradually going all the way across from bismuth to antimony. And then David Egger’s group may calculate what this will do to the masses of the charges, which may predict whether they should be more mobile or not. Our experiments in my group may then examine whether that really reflects the truth. And the answer may be that it’s not such a simple story, that it also could have something to do with how easily these materials crystallize. Then we might go back, passing it back to the synthetic chemists, and say hey, you have to find better ways of making that crystallization work. So that’s an example of one particular feedback loop.
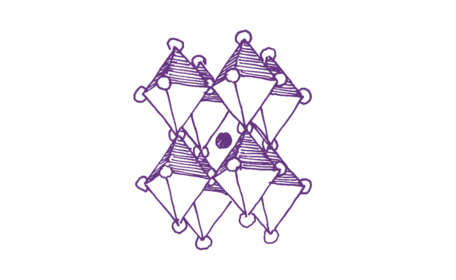
Q: I understand that device design, system development, manufacturing, and the electrical grid are outside your research focus, but you must have a sense of where these are heading with respect to perovskite solar cells. What can you say about the outlook
I’d say it’s now at the pilot plant stage. For example, the spin-out company Oxford Photovoltaics, which was founded in 2010, has bought and repurposed a factory in Brandenburg, Germany, to produce prototype perovskite solar cells. There are also companies in the United States trying hard to bring a product to market. I think the first product to market probably would be perovskite on silicon, because silicon is well established, and it would be seen as a booster layer on top of silicon. Such perovskite-silicon tandem cells have already demonstrated lab-based power conversion efficiencies above 30 percent. So that is an exciting concept, and stability, another important aspect, is looking promising as well.
Q: How or why did researchers first decide to try using metal-halide perovskites for energy conversion? Was it a hint from theory or a hunt through libraries of materials?
I think it was more of a hunt through libraries of materials. Tsutomu Miyasaka in Japan tried to use this material, essentially as a sensitizer, in dye-sensitized mesoporous metal-oxide solar cells. He tried using lead iodide perovskite as a replacement for the dye, and it didn’t work well for long. It showed some decent efficiency, but the perovskite quickly decomposed in the electrolyte forming part of this type of solar cell. Then Henry Snaith at Oxford talked to him, and they came up with the idea of using the perovskite to actually replace the electrolyte, to make an all-solid-state perovskite solar cell, still incorporating mesoporous metal oxides. Michael Johnston, here at Oxford, evaporated the material for the first time and showed that this thin-film layer works just as well, even without the metal oxide. In the beginning, it really was serendipity, but as usual, progress relied on many different contributions from researchers around the world.